SUBMILLIMETER
WAVE
Color |
Frequency |
Wavelength |
Violet |
668–789 THz |
380–450 nm |
Blue |
606–668 THz |
450–495 nm |
Green |
526–606 THz |
495–570 nm |
Yellow |
508–526 THz |
570–590 nm |
Orange |
484–508 THz |
590–620 nm |
Red |
400–484 THz |
620–750 nm |
|
Submillimeter
Wave :
Submillimeter
wave astronomy is a relatively new branch of astronomy that studies
celestial objects using the submillimeter band of the electromagnetic
spectrum, which ranges from 0.1 mm to 1.0 mm (300 GHz to 3000 GHz).
This band, which lies between the far infrared and high-frequency
radio bands, contains valuable astonomical information in both continuum
and molecular spectral lines, but has been unavailable to astronomers
until recently because most of the radiation is blocked by the Earth's
atmosphere. In order to overcome this barrier, submillimeter observatories
are usually placed at high altitude.
Band
8 (385 to 500 GHz, submillimeter-wave)
125000
/ 108 (beads in mala) = 1157.4074
1157.4074
/ 3 (waves) = 385.80246
385.80246
submillimeter wave
Band
8 (385 to 500 GHz, submillimeter-wave)
Using
submillimetre observations, astronomers examine molecular clouds
and dark cloud cores with a goal of clarifying the process of star
formation from earliest collapse to stellar birth.
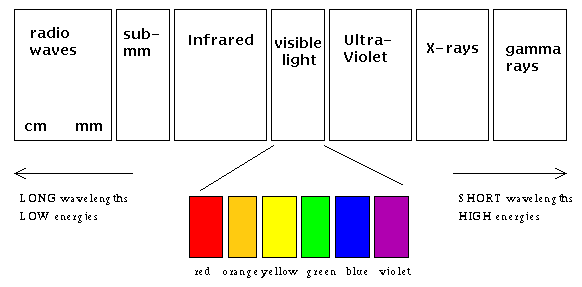
In
our EVeryday human experience, we see that light has measurable
properties. It has intensity (brightness), and it has color. The
intensity gives an indication of the number of light "waves"
or "particles" (called photons) coming from an object.
The color is a measure of the energy contained in each photon. The
colors of the rainbow (red, orange, yellow, green, blue, violet)
denote the energies of light waves that our human eyes can see and
interpret. This "color" or "energy" range is
called the visible spectrum. Red photons of light have the least
energy, violet photons carry the most energy. Until fairly recently,
all of our astronomical knowledge came from the detailed study of
visible light from astronomical objects.
The
visible spectrum is only a tiny portion of the total electromagnetic
(EM) spectrum, however.
Notice
that radio, TV, and microwave signals are all light waves, they
simply lie at wavelengths (energies) that your eye doesn't respond
to. On the other end of the scale, beware the high energy UV, x-ray,
and gamma-ray photons! Each one carries a lot of energy compared
to their visible- and radio-wave brethren. They're the reasons you
should wear sunblock, for example.
When
we look at the Universe in a different "light", i.e. at
"non-visible" wavelengths, we probe different kinds of
physical conditions -- and we can see new kinds of objects! For
example, high-energy gamma-ray and X-ray telescopes tend to see
the most energetic dynamos in the cosmos, such as active galaxies,
the remnants from massive dying stars, accretion of matter around
black holes, and so forth. Visible light telescopes best probe light
produced by stars. Longer-wavelength telescopes best probe dark,
cool, obscured structures in the Universe: dusty star-forming regions,
dark cold molecular clouds, the primordial radiation emitted by
the formation of the Universe shortly after the Big Bang. Only through
studying astronomical objects at many different wavelengths are
astronomers able to piece together a coherent, comprehensive picture
of how the Universe works.
Submillimeter-wavelength
(0.3 - 1.0 mm) astronomy is perhaps the last wholly unexplored wavelength
frontier. Why? Submillimeter ("microwave") astronomy is
technically very difficult due to the sheer complexity of the instrumentation
and to the "opaqueness" of the atmosphere in microwave
light.
The
Submillimeter (a.k.a. "microwave") frequency band lies
between the regions easily observed by radio telescopes and optical
telescopes. It comes as no surprise, then, that submillimeter astronomy
borrows techniques used by both optical and radio astronomers. The
visual appearance and operation of the telescope is that of a radio
telescope, although it is sheltered in an enclosure like an optical
telescope. Continuum-mode observations are done using specialized
heat-sensing detectors called bolometers, which stem from infrared
techniques. Spectral-line measurements, incorporating very high
wavelength-resolution, use heterodyne receivers somewhat resembling
those found in lower-frequency radio receivers. Such high frequency
receivers (approaching 1 Terahertz, about 10,000 times higher frequency
than the average FM radio!) are very difficult to manufacture, howEVer.
The recent production of sensitive receivers for astronomical purposes
(at the University of Arizona (SORAL), among other places) has led
recently to the opening of this wavelength band for the first time.
Ground-based
submillimeter observations are difficult for another reason: the
opaque-ness (opacity) of the atmosphere at microwave wavelengths.
Here's
another (prettier) plot of the electromagnetic spectrum. The different
color-coded classifications reflect segments of the EM spectrum
which require different techniques for observation.
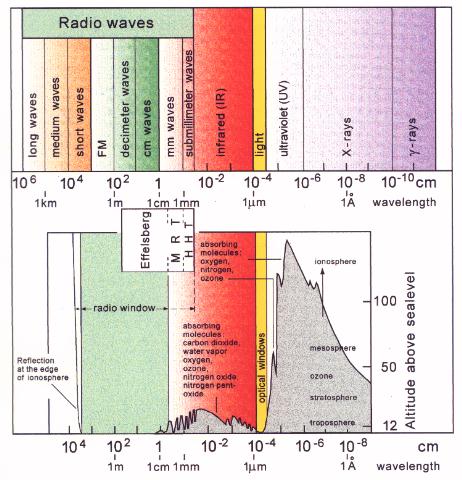
The
lower plot focuses on a portion of the spectrum from radio wavelengths
to x-rays. The gray-filled line demonstrates at what height in the
Earth's atmosphere incoming waves of light are blocked. Notice that
the Earth's atmosphere blocks high-energy light (UV, X-rays, Gamma
rays) dozens of miles above our heads. To observe astronomical X-ray
sources, one must therefore launch astronomical satellites above
the atmosphere.
At
the opposite extreme, most radio- and visible- wavelength observations
are unimpeded by the atmosphere; the incoming photons can travel
right through. The atmosphere is transparent at such wavelengths.
Transparency is important since astronomers must be able to look
through the atmosphere to observe astronomical objects.
At
submillimeter wavelengths, ambient atmospheric water vapour will
absorb (block) incoming light. At low elevations, where most water
vapour resides, the atmosphere is very opaque at submillimeter wavelengths;
the abundant water vapour absorbs any incoming submillimeter photons
before they can reach the telescope. At higher elevations, however,
the water content decreases substantially. By minimizing the atmospheric
water vapour, one improves the transparency of the atmosphere and
makes astronomical observations possible. It is for this reason
that infrared and submillimeter observatories are built as high
as possible; by being above some of the atmosphere, the radiation
from astronomical sources is much less attenuated.
To
demonstrate how important a dry site is to a submillimeter observatory,
look at the following plot :
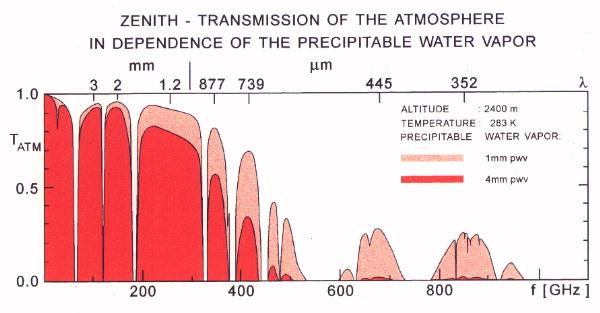
The
transparency (transmission efficiency) of the atmosphere at microwave
frequencies is plotted for two amounts of atmospheric water: 1 mm
and 4 mm of precipitable water vapour. This term deserves an explanation:
4 mm of PWV means that if EVery molecule of water vapour above you
could be condensed into an ocean, it would be 4 mm deep! 4 mm of
water vapour is in fact still pretty dry, but EVen there, the atmosphere
blocks all but 2% of the light at 0.3 mm (800 GHz). Moved to a very
high, dry site under excellent conditions, you might expect to see
1 mm of PVW; and the atmosphere would pass about 25% of the light
at 0.3 mm. Lower frequency observations (below 200 GHz, a.k.a "millimeter"-wave
astronomy) can be performed under conditions when submillimeter
observations are impractical or impossible.
Submillimeter
astronomy promises to yield a new view upon the Universe we live
in, almost certainly shedding light upon many of the outstanding
questions in modern astronomy.
Among
the most pressing of these questions is how stars are formed. Stars
are born from the material between other stars. In some regions
of space, the density of gas and dust is much higher than the norm,
and atoms are sufficiently shielded from destructive high-energy
photons to interact with other atoms to form simple molecules. In
the highest density central regions of such molecular clouds, material
is so well shielded that delicate, complex molecules can form. It
is from these molecular cloud cores that new stars (including our
own Sun) are born. The study of the chemistry in such regions can
not only lead to an improved understanding of the physical conditions
in such a "stellar nursery", but also can provide clues
to the composition of other star-forming (and possibly planet-forming
and life-giving) solar systems. Such studies may help astronomers
understand the conditions from which life evolved on Earth.
Because
the star forming process occurs behind so much intervening dusty
material, visible-light telescopes cannot see what is happening.
By moving to the infrared, sub-millimeter and millimeter wavelength
regions, where the effects of this obscuration are nearly negligible,
astronomers can begin to directly probe regions where stars are
actively being born.
Understanding
the star forming process has many astronomical consequences. Knowing
what physical conditions are needed to form molecular cloud complexes
is important in understanding the star-forming EVolution of galaxies,
both in the current age and, perhaps most importantly, when galaxies
were first forming. The understanding of how a single star forms
from a molecular cloud, then, has EVen cosmological implications.
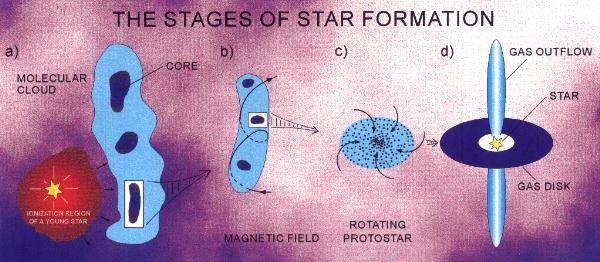